Introduction
Cerebral ischemia in intracranial surgery can lead to postoperative motor deficits and fresh cerebral infarctions that can significantly increase mortality risk [1]. Motor deficits following neurosurgical procedures cause significant morbidity and mortality and increased medical costs associated with extended length of stay and rehabilitation [2]. Moreover, temporary occlusion without adequate collateral circulation causes cerebral hypoperfusion [3]. Intraoperative neurophysiological monitoring (IONM) using somatosensory evoked potentials (SEPs) can detect reduced cerebral blood flow to allow intraoperative adjustments to restore and improve blood flow and subsequently reverse the changes in SEPs [3]. Reduced SEP amplitude and cerebral blood flow are closely related; therefore, SEPs are monitored to detect cerebral ischemia [4]. This process involves monitoring the functional integrity of neural pathways and mapping techniques to identify and preserve the cranial nerves, their motor nuclei, and corticospinal or corticobulbar pathways during posterior-fossa and brainstem surgery [5]. Motor evoked potentials (MEPs) provide superior sensitivity and specificity to SEPs in detecting functional disturbances in the pyramidal motor pathways [6]. Monitoring changes in SEPs lacks sensitivity to detect subcortical ischemia caused by disrupted blood flow in the deep perforating arterial branches [7]. However, SEP and MEP monitoring is the gold standard for real-time functional observation of the corticospinal tract during tumor surgery [8]. To minimize neurological damage during surgery, IONM is used to identify important neural structures in the operative field to avoid and limit significant postoperative impairments [9].
Intraoperative monitoring of SEPs and MEPs during brain surgery is important to predict postoperative motor deterioration. This study aimed to identify the cutoff points at which changes in SEPs and MEPs were the most reliable indicators of motor deterioration after brain surgery.
Materials and Methods
This retrospective observational study of intraoperative SEPs or MEPs included 104 inpatients admitted from the clinic of the department of rehabilitation at a tertiary hospital between December 2015 and December 2016. All operations were performed by highly experienced neurosurgeons. Patient data including demographic characteristics, operative narratives and IONM, medical, anesthesia, and outpatient clinical records were extracted from electronic medical records. Preoperatively, 80 patients were neurologically intact and 24 exhibited motor deterioration. Their baseline characteristics are listed in Table 1.
Rocuronium bromide (Esmeron®, 50–150 mg, MSD, Seoul, Korea) was administered intravenously as a short-acting muscle relaxant to facilitate endotracheal intubation. No paralytic agents were subsequently administered. General anesthesia was induced by total intravenous anesthesia (TIVA) using combinations of remifentanil (Ultiva®, Mitsubishi Tanabe Pharma Korea, Seoul, Korea), propofol (Fresofol®, Fresenius-Kabi Korea, Seoul, Korea), or midazolam (Vascam®, Hana Pharm, Seoul, Korea). During anesthesia, body temperature, direct radial artery pressure, pulse rate, oxygen saturation, and end-tidal carbon dioxide concentration were continuously monitored. All patients were kept normothermic and normotensive.
IONM was performed by a single skilled technician using a Cascade system (Cadwell Industries, Kennewick, WA, USA). Both SEPs and MEPs were monitored in all 104 patients.
SEPs were elicited by electrical stimulation of the median nerve at the wrist and posterior tibial nerve at the ankle (40 mA intensity, 0.2 ms duration, 5 Hz repetition rate). SEPs were recorded using needle electrodes placed on the scalp at C3 (right median nerve), C4 (left median nerve), and Cz (right or left tibial nerve) referenced to Fpz according to the 10–20 international EEG system.
MEPs were obtained by administering multi-pulse transcranial electric stimulations using a Cascade electrical stimulator (Cadwell Industries). Transcranial electric MEPs were recorded bilaterally from the abductor pollicis brevis muscles in the upper extremities and tibialis anterior muscles in the lower extremities using a pair of needle electrodes inserted 3 cm apart in each muscle. Short trains of six square-wave stimuli (0.5 ms duration, 5 ms interstimulus interval, up to 2 Hz repetition rate) were delivered through needle electrodes placed at C1 and C2, according to the 10–20 international EEG system. A C1/C2 montage was preferable for right-extremity MEPs, while C2/C1 was preferentially used to elicit left-extremity MEPs. To elicit lower-extremity MEPs, a Cz/Fz montage was used, which produced less intense muscle twitching. The stimulus intensity was gradually escalated by 50 mV increments (from 50 mV to 400 mV) until the MEP amplitudes were maximized above a minimum of 10 mV.
Neurophysiological monitoring was performed throughout the surgical procedures. Baseline EPs were obtained at least 60 minutes after intubation and after the muscle relaxant effect had faded. The N20 latencies of the median SEPs, P37 latencies of tibial SEPs, and peak-to-peak amplitudes of MEPs were continuously monitored. More than 50% reduction in amplitude compared to baseline MEPs and prolongation of N20 or P37 latencies by >10% from baseline SEPs were defined as significant changes. If any significant change in EPs occurred, surgeons were promptly informed and surgical procedures were stopped temporarily until the values returned to normal. However, if no signal reversal occurred even after surgical correction immediately before the signal change, the procedure was stopped.
The motor status of each patient was evaluated before surgery and 48 h and 4 weeks post-surgery. The strengths of 10 key muscles were measured using the Medical Research Council (MRC) scale ranging from 0 to 5. The total score ranged from 0 to 50 points on each side. We used the sum of MRC scale of both extremities for analysis ranging from 0 to 100. Any reduction in the motor score by ≥1 point compared to the preoperative evaluation was considered postoperative neurological motor deterioration. Postoperative weakness observed within 48 h after surgery, which recovered after 4 weeks, was considered transient motor deterioration. A decreased motor score at 48 h that did not recover in 4 weeks was considered persistent postoperative motor deterioration. Statistical analysis was performed to reveal the correlations between the intraoperative changes in SEPs or MEPs and motor status according to each brain disease type.
Correlation analysis was used to determine the associations between changes in SEPs and MEPs. Sensitivity, specificity, positive predictive value (PPV), and negative predictive value (NPV), used in previous studies as the alarm criteria to predict postoperative motor deterioration, were calculated. To maximize the sensitivity values, receiver operating characteristic (ROC) curve analysis was performed, followed by analyses of the sensitivity, specificity, PPV, and NPV of the best cutoff point. Mann–Whitney U tests were performed to compare continuous values. Data were analyzed using IBM SPSS Statistics for Windows, version 20.1 (IBM, Armonk, NY, USA). p-values <0.05 were considered statistically significant.
Results
A total of 104 patients were enrolled. Their baseline characteristics are presented in Table 1. The median age was 58.5 years (range 7–83 years) and 58 (55.8%) patients were women. The average preoperative and postoperative motor scores (MRC scale) were 96.4 and 94.6, respectively. A large proportion of patients (63 patients) had vascular disease, with un-ruptured aneurysm (41 patients) the most common. Moreover, eight patients with ruptured aneurysm/SAH and eight with carotid stenosis were included. The study included 41 patients with tumor disease, with meningioma the most common (16 patients). Patients with glioblastoma, astrocytoma, and craniopharyngioma were also included. The other diseases included glioma, chordoma, choroid plexus papilloma, pineal gland tumor, and papillary glioneuronal tumor.
Of the 104 patients, 12 showed postoperative motor deterioration. Baseline motor scores were evaluated using the MRC scale. Eighty and 24 patients were analyzed on 100-point and <100-point scales, respectively. Among patients with motor intact at baseline, 95% exhibited no change after brain surgery, while 5% exhibited motor deterioration. However, 33.3% of patients with baseline motor deficits showed postoperative motor deterioration.
The characteristics of the 12 patients with postoperative motor deterioration are described in Table 2. All patients had persistent motor deterioration after brain surgery. Among these patients, only one had vascular disease (ruptured cerebral aneurysm); among the other patients, tumor disease and meningioma were the most common disease types. Eight patients exhibited changes in both SEPs and MEPs, none showed changes in SEPs alone, and three showed changes in MEPs alone. One patient showed changes neither in SEPs nor MEPs.
In addition to preoperative SEPs and MEPs, intraoperative EPs were measured and the changes in SEPs and MEPs were calculated. The median change in SEP latency delay (ΔSEPLat) was defined as the difference between the latency of the preoperative SEPs and the latency of the most delayed SEPs during surgery. The reduction in MEP amplitude (ΔMEPAmp) was defined as the difference between the amplitude of preoperative MEPs and the amplitude of the most decreased MEPs during surgery. The ΔSEPLat and ΔMEPAmp were 4.9% and 21.2%, respectively. Subgroup analysis of ΔSEPLat and ΔMEPAmp to predict postoperative motor deterioration based on the disease type showed median ΔSEPLat and ΔMEPAmp of 4.7% and 20.2% in the vascular group and 5.9% and 25.4%, respectively, in the tumor group. Both ΔSEPLat and ΔMEPAmp were more prominent in the tumor group than in the vascular group. All values of each parameter are shown in Table 3. We observed no significant association between ΔSEPLat and ΔMEPAmp (p = 0.29). ΔMEPAmp was more prominent than ΔSEPLat in both groups (Fig. 1).
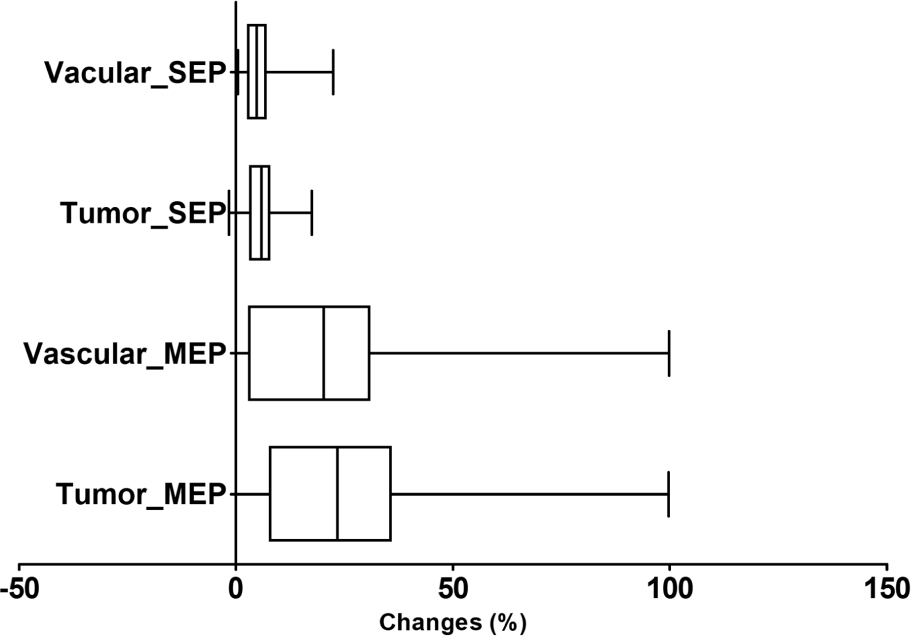
The sensitivity and specificity of using the previous alarm criteria (ΔSEPLat >10% delay) were 8.3% and 96.7%, while those for ΔMEPAmp (>50% reduction) were 16.7% and 95.7%, respectively. The sensitivity of patients with ΔSEPLat >10% or ΔMEPAmp >50% increased from 8.3% to 25%, while specificity decreased from 96.7% to 93.5%. The PPV and NPV were also evaluated based on the previous alarm criteria. The PPV and NPV for ΔSEPLat >10% alone were 25% and 89%, respectively, while the corresponding values for ΔMEPAmp alone were 33.3% and 89.8%. The prediction of postoperative motor deterioration using ΔSEPLat >10% or ΔMEPAmp >50% yielded PPV and NPV of 33.3% and 90.5%, respectively.
As the sensitivity was low using the previous alarm criteria, we identified new alarm criteria using ROC curve analysis. Based on this analysis, changes of 7.1% and 21% were the best points for ΔSEPLat and ΔMEPAmp, respectively (Fig. 2). The same results were obtained in 41 patients who underwent brain tumor surgery.
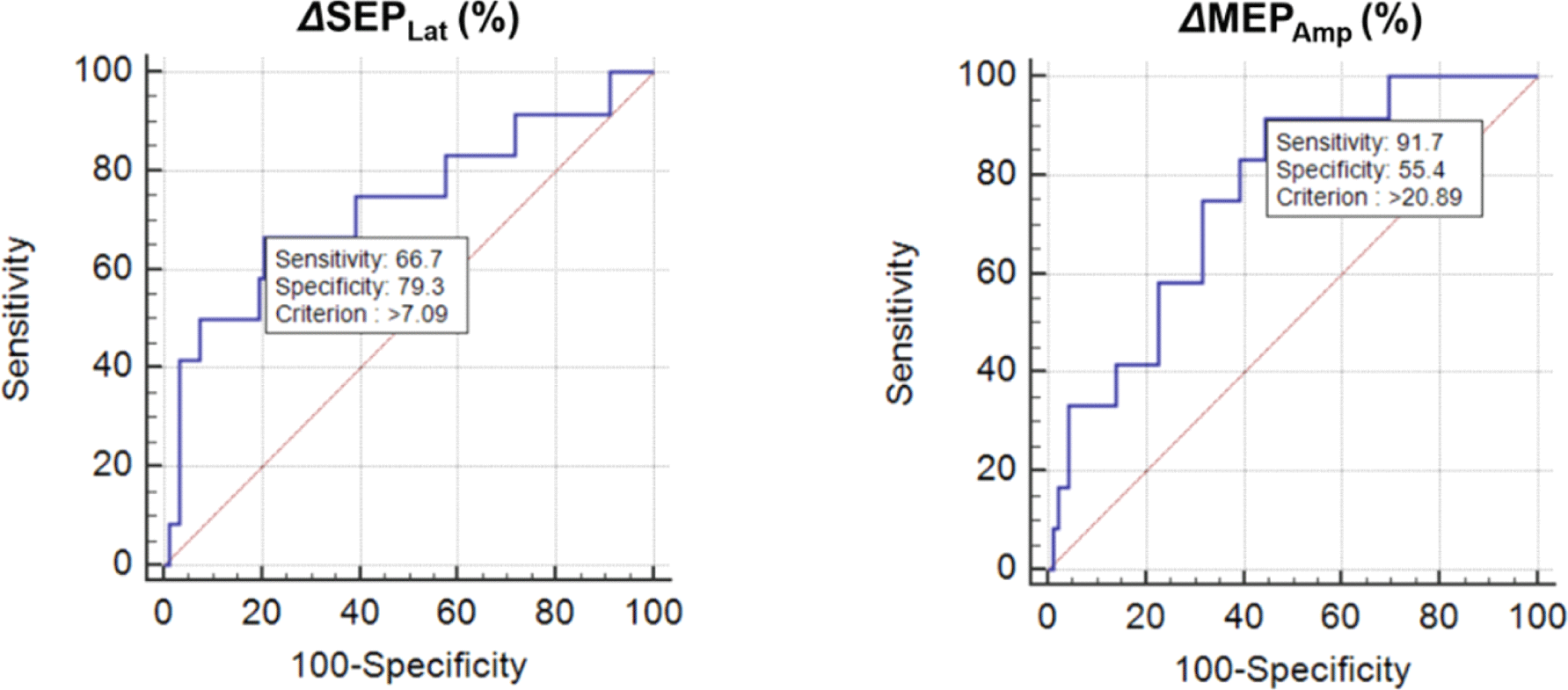
After identifying the new cutoff, we evaluated its sensitivity, specificity, PPV, and NPV for the prediction of postoperative motor deterioration. The sensitivity and NPV of both SEPs and MEPs improved significantly compared with those of the previous alarm criteria. The sensitivity of ΔSEPLat >7.1% alone was 66.7% and that for ΔMEPAmp >21% alone was 91.7%. We observed a corresponding reduction in the specificity and PPV of each parameter.
Subgroup analyses were also performed and the reliability of EPs using the previous alarm criteria and the new cutoff were evaluated. The sensitivity and specificity in the tumor disease group were 27.3% and 90%, respectively, for ΔSEPLat >10% or ΔMEPAmp >50%. The sensitivity and NPV of SEPs and MEPs when using the new alarm criteria were significantly improved compared to those of the previous alarm criteria. The sensitivity and specificity of ΔSEPLat >7.1% or ΔMEPAmp >21% were 90.9% and 46.7%, respectively in the brain tumor disease group. The corresponding values for the specificity of each parameter decreased. We observed increased PPV and NPV with the new cutoff criteria for MEPs alone. The values corresponding to each parameter are shown in Table 4.
Discussion
Monitoring both SEPs and MEPs provides a wide range of information during brain surgery. Among 104 patients monitored in this study, 12 experienced postoperative neurological weakness characterized as permanent neurological deterioration, 11 of whom had brain tumors as the initial reason for brain surgery. In both vascular and tumor groups, the range of ΔMEPAmp was larger than that in ΔSEPLat. However, this difference was not significant. Based on the current literature, the pre-existing cutoff value was defined as a ΔSEPLat of >10% or a ΔMEPAmp of >50%. With these criteria, the sensitivity in detecting impending neurologic deteriorations after brain surgery was 25% while the specificity was 93.5%. EPs monitoring using the new cutoff alarm criteria yielded a sensitivity of 91.7% and specificity of 42.4% in detecting impending neurologic deteriorations after brain surgery. The results were the same for patients with brain tumors only.
In the tumor group, sensitivity and specificity were 27.3% and 90%, respectively, for the pre-existing alarm criteria, and 90.9% and 46.7% for the new cutoff point. For MEPs monitoring, the PPV and NPV were 40% and 75% for the pre-existing alarm criteria, and 45.5% and NPV being 94.7% for the new cutoff point. With the new cutoff value, the NPV increased for the monitoring of both SEPs and MEPs.
However, increasing only the sensitivity during surgery may be a problem in providing feedback to the operator during the operation. Therefore, more diverse solutions should be considered for practical application. Our results were obtained after adjustments were made owing to monitoring during surgery, which may have influenced the postoperative results. When the cutoff point was changed in patients with brain tumors, the sensitivity increased but the specificity decreased because the threshold was lowered. Thus, at least for patients with brain tumors, a value different from the conventional cutoff value can be applied.
The use of SEPs is advantageous because these do not provoke unwanted patient movement during surgery and are easily quantifiable [9]. Moreover, SEPs may avoid false-negative findings arising from high-intensity MEP stimulation used to activate sites deep in the cortex [10]. SEPs provide direct information about somatosensory regions and pathways and assist in monitoring when MEPs cannot be obtained from patients with significant preoperative weakness [11]. SEPs are also useful in avoiding nerve injuries associated with limb, neck, and head positioning [12].
However, SEPs are sensitive only to cortical mantle ischemia in the region around the middle cerebral artery [3]. Monitoring of SEPs is typically insensitive to strokes in the rostral frontal, temporal, and occipital lobes and to subcortical ischemia, particularly that occurring secondary to perforating vessel perturbation [3].
The benefits of IONM, especially the monitoring of MEPs, in avoiding direct damage to the corticospinal tract during surgery for supratentorial lesions have been demonstrated [13]. The relationships between MEP loss and permanent postoperative neural deficit during aneurysm occlusion of basilar, vertebral, and middle cerebral artery aneurysms have been demonstrated [14]. MEPs may also be more sensitive and show changes faster than SEPs in brainstem ischemia caused by perforating artery occlusion during basilar tip aneurysm surgery [15]. Lesions outside the primary motor cortex or pyramidal tract, such as the basal ganglia, and thalamus or hemorrhage extending to the center of the somatosensory cortex and intraparietal sulcus, which are not associated with MEP detection, could similarly interfere with motor function recovery [16-18]. Thus, the monitoring of MEPs is useful during brain tumor removal [19-22].
The combination of MEPs and SEPs monitoring during surgery for intraparenchymal and extraparenchymal brainstem lesions has emerged as a safe, reliable, and sensitive method to detect and reduce brainstem injury, thereby allowing early interventions to avoid permanent impairment [23]. Monitoring SEPs/MEPs in intracranial aneurysm surgery is of increasing interest among surgeons for detecting and correcting the risk of intraoperative ischemic [24]. SEPs provide an established modality for monitoring somatosensory pathway function during surgery on the spinal cord, brain, and brainstem to detect iatrogenic neurological injury, an effective indicator of brainstem integrity. However, SEPs are sensitive to anesthetic agents; thus, anesthetics may interfere with SEP recording [25].
Although MEPs can be monitored under the standard TIVA protocol, they are highly vulnerable to inhalation anesthesia such that even a low dose of halogenated inhalation anesthetics can abolish or significantly interfere with MEPs recording [26]. Recording requires the transmission of a neural signal through the neuromuscular junction; thus, the use of muscle relaxants (neuromuscular blockers) during surgical procedures can significantly affect the MEP amplitude [27]. In our study, we did not observe any statistically significant correlation between ΔSEPLat and ΔMEPAmp. However, patients with motor deterioration experienced greater ΔMEPAmp. It is difficult to determine whether these new deficits occurred due to intraoperative ischemic events or technical errors in monitoring SEPs, or delayed postoperative ischemic events, which would be impossible to recognize in SEP monitoring [3].
Nonetheless, monitoring SEPs and MEPs in our study has some limitations. The most important limitation was the heterogeneity of the patient group. We included both brain tumor and brain vascular disorder group despite of their differences in operation methods and mechanism of EP compromise pattern. These could have interfered the result of this study and further investigation classifying patients according to specific diseases is required to figure out more precise cut-off value. Moreover, our study did not reflect patients’ underlying diseases or conditions. We also defined ‘motor weakness’ as a single point or more decrease on the MRC scale. Therefore, we included even mild weakness, which presumably also affected the sensitivity and specificity. An additional limitation was that we did not consider sensory deficits and assessed only motor outcomes. The follow-up conducted 1 month later was also a limitation. Additional follow-ups at 6 and 12 months would allow better interpretations of the benefits of monitoring SEPs and MEPs. Multimodal monitoring provides surgeons more complete neurological information, including additional information regarding risk factors, and further enhances sensitivity.
Because of the factors listed above, it is clinically hard to use the criteria presented in this study. However, this study is meaningful to suggest that the current established criteria is low in sensitivity, and may also need to be revised. Further studies should find most suitable criteria that can increase both sensitivity and specificity.
Conclusion
Intraoperative SEP and MEP monitoring is highly specific for predicting impending stroke and neurologic deterioration after brain surgery. IONM is increasingly widespread in recent years. The sensitivity and specificity of intraoperative SEP and MEP monitoring may differ by surgical procedure and location of the brain lesion. Brain tumor and cerebrovascular surgeries have different results and IONM values owing to their different approaches. Therefore, appropriate monitoring methods are needed for different surgical approaches such as those for cerebrovascular or brain tumor resection.